Research Projects
Exploring Brain Mechanics (EBM):
Understanding, engineering and exploiting mechanical properties and signals in central nervous system development, physiology and pathology (projects A01 and B01)
(Third Party Funds Group – Overall project)
Term: 1. January 2023 – 31. December 2026
Funding source: DFG / Sonderforschungsbereich / Transregio (SFB / TRR)
The central nervous system (CNS) is our most complex organ system. Despite tremendousprogress in our understanding of the biochemical, electrical, and geneticregulation of CNS functioning and malfunctioning, many fundamental processesand diseases are still not fully understood. For example, axon growth patterns inthe developing brain can currently not be well-predicted based solely on thechemical landscape that neurons encounter, several CNS-related diseases cannotbe precisely diagnosed in living patients, and neuronal regeneration can stillnot be promoted after spinal cord injuries.
Duringmany developmental and pathological processes, neurons and glial cells aremotile. Fundamentally, motion is drivenby forces. Hence, CNS cells mechanicallyinteract with their surrounding tissue. They adhere to neighbouring cells and extracellular matrix using celladhesion molecules, which provide friction, and generate forces usingcytoskeletal proteins. These forces aretransmitted to the outside world not only to locomote but also to probe themechanical properties of the environment, which has a long overseen huge impacton cell function.
Onlyrecently, groups of several project leaders in this consortium, and a few other groupsworldwide, have discovered an important contribution of mechanical signalsto regulating CNS cell function. For example, they showed that brain tissuemechanics instructs axon growth and pathfinding in vivo, that mechanicalforces play an important role for cortical folding in the developing humanbrain, that the lack of remyelination in the aged brain is due to an increasein brain stiffness in vivo, and that many neurodegenerative diseases areaccompanied by changes in brain and spinal cord mechanics. These first insights strongly suggest thatmechanics contributes to many other aspects of CNS functioning, and it islikely that chemical and mechanical signals intensely interact at the cellularand tissue levels to regulate many diverse cellular processes.
The CRC 1540 EBM synergises the expertise of engineers, physicists,biologists, medical researchers, and clinicians in Erlangen to explore mechanicsas an important yet missing puzzle stone in our understanding of CNSdevelopment, homeostasis, and pathology. Our strongly multidisciplinary teamwith unique expertise in CNS mechanics integrates advanced invivo, in vitro, and in silico techniques across time(development, ageing, injury/disease) and length (cell, tissue, organ) scalesto uncover how mechanical forces and mechanical cell and tissue properties,such as stiffness and viscosity, affect CNS function. We especially focus on(A) cerebral, (B) spinal, and (C) cellular mechanics. Invivo and in vitro studies provide a basic understanding ofmechanics-regulated biological and biomedical processes in different regions ofthe CNS. In addition, they help identify key mechano-chemical factors forinclusion in in silico models and provide data for model calibration andvalidation. In silico models, in turn, allow us to test hypotheses without the need of excessive or even inaccessibleexperiments. In addition, they enable the transfer and comparison of mechanics data and findingsacross species and scales. They also empower us to optimise processparameters for the development of in vitro brain tissue-like matricesand in vivo manipulation of mechanical signals, and, eventually, pavethe way for personalised clinical predictions.
Insummary, we exploit mechanics-based approaches to advance ourunderstanding of CNS function and to provide the foundation for futureimprovement of diagnosis and treatment of neurological disorders.
Recent evidence suggests that mechanical forces drive cortical folding during brain development. While analytical, computational, and experimental models have significantly advanced our understanding of the mechanisms underlying the folding process, such models have so far not been used to tackle specific clinical challenges. For example, folding patterns are an important clinical hallmark of cortical development and brain malformations, such as those related to epilepsy. Assisting clinicians in the diagnosis and treatment of brain folding-related neurological disorders, e.g., by reverse-engineering the cellular processes that could have led to the macroscopic malformation, requires the close collaboration between clinicians, who define open clinical questions, and engineers, who develop targeted simulation tools to address them. To tackle some of the open questions concerning malformations associated with epilepsy analyzed in A02, we plan to use mechanical modelling approaches. A01 aims to develop a computational framework to numerically predict the mechanisms underlying abnormal brain development. Based on qualitative and quantitative insights into the interplay between mechanics, cell migration, cell differentiation, and brain malformations obtained through projects A02 through A05, we will establish a multifield theoretical framework to predict brain development under physiological and pathological conditions. For model calibration and validation, we will use the various human data sets generated in project A02 supplemented by mechanical tests under compression, tension, and torsional shear on fresh human brain tissue obtained from neurosurgical procedures. We will incorporate data from patients into the modelling framework to lay the foundation for later use in clinical practice – to advance from benchmark problems to disease-specific predictions and eventually assist the diagnosis and treatment of neurological diseases such as epilepsy.
B01 aims to establish a continuum-based computational framework to predict the regeneration of spinal cord tissue after injury or disease. The computational model will capture the temporal and spatial evolution of growth, remodelling and healing processes, as experimentally observed in B02-B05. We will specifically focus on mechanics-driven processes that are involved in the regeneration of the spinal cord after traumatic injury and in multiple sclerosis. We will capture the evolving connectivity of cells in the central nervous system by continuous order parameters driven by mechanics and biochemical factors. To calibrate the constitutive models, we will exploit mechanical tests on human and animal spinal cord tissue performed in B01-B05. Modelling in B01 will help correlating the comprehensive set of ex vivo and in vivo mechanical data, data from various species, and multiple measurement techniques within EBM. B01 will in particular enter into a close feedback loop with B03, which provides data on in vivo tissue mechanics based on Brillouin microscopy (BM) measurements and ex vivo tissue mechanics based on atomic force microscopy (AFM) measurements and correlated structural and compositional information. B01 will in turn provide information about mechanical determinants identified through modelling and simulation. B01 will furthermore provide testable hypotheses for targeted experiments in B03 and the associated results will be directly fed back into our computational framework.
Biofabricated gradients for functional tissue models (B09*)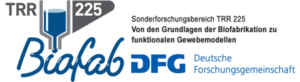
(Third Party Funds Group – Sub project)
The aim of this project is to develop a platform technology to produce gradients that are clearly defined and reproducible in space and time, to analyze them and to model them in silico in order to be able to investigate their effect on cell-biomaterial interactions. To this end, we will first develop printheads that can be used to generate controlled transitions of materials from the A/B projects, drugs, and cells. Through comprehensive characterization of the printed gradients using mechanical test methods in combination with imaging techniques, the results will be continuously analyzed and improved with respect to the requirements of the C-projects. In addition, continuum mechanical modeling and simulation are used specifically to optimize process parameters, the print pattern and the 3D arrangement in the construct.
BRAIn mechaNIcs ACross Scales: Linking microstructure, mechanics and pathology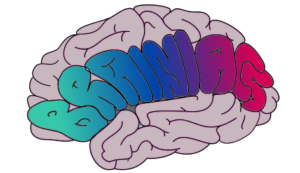
(Third Party Funds Single)
Funding source: DFG-Einzelförderung / Emmy-Noether-Programm (EIN-ENP)
URL: https://www.brainiacs.forschung.fau.de/
The current research project aims to develop microstructurallymotivated mechanical models for brain tissue that facilitate early diagnosticsof neurodevelopmental or neurodegenerative diseases and enable the developmentof novel treatment strategies. In a first step, we will experimentallycharacterize the behavior of brain tissue across scales by using versatiletesting techniques on the same sample. Through an accompanying microstructuralanalysis of both cellular and extra-cellular components, we will evaluate thecomplex interplay of brain structure, mechanics and function. We will alsoexperimentally investigate dynamic changes in tissue properties duringdevelopment and disease, due to changes in the mechanical environment of cells (mechanosensing),or external loading. Based on the simultaneous analysis of experimental andmicrostructural data, we will develop microstructurally motivated constitutive lawsfor the regionally varying mechanical behavior of brain tissue. In addition, wewill develop evolution laws that predict remodeling processes duringdevelopment, homeostasis, and disease. Through the implementation within afinite element framework, we will simulate the behavior of brain tissue underphysiological and pathological conditions. We will predict how known biologicalprocesses on the cellular scale, such as changes in the tissue’smicrostructure, translate into morphological changes on the macroscopic scale,which are easily detectable through modern imaging techniques. We will analyzeprogression of disease or mechanically-induced loss of brain function. The novelexperimental procedures on the borderline of mechanics and biology, togetherwith comprehensive theoretical and computational models, will form thecornerstone for predictive simulations that improve early diagnostics of pathologicalconditions, advance medical treatment strategies, and reduce the necessity ofanimal and human tissue experimentation. The established methodology will furtheropen new pathways in the biofabrication of artificial organs.
Novel Biopolymer Hydrogels for Understanding Complex Soft Tissue Biomechanics
(FAU Funds)
URL: https://www.biohydrogels.forschung.fau.de/
Biological tissues such as blood vessels, skin, cartilage or nervous tissue provide vital functionality
to living organisms. Novel computational simulations of these tissues can provide insights
into their biomechanics during injury and disease that go far beyond traditional approaches. This
is of ever increasing importance in industrial and medical applications as numerical models will
enable early diagnostics of diseases, detailed planning and optimization of surgical procedures,
and not least will reduce the necessity of animal and human experimentation. However, the extreme
compliance of these, from a mechanical perspective, particular soft tissues stretches conventional
modeling and testing approaches to their limits. Furthermore, the diverse microstructure
has, to date, hindered their systematic mechanical characterization. In this project, we will, as a
novel perspective, categorize biological tissues according to their mechanical behavior and identify
biofabricated proxy (substitute) materials with similar properties to reduce challenges related
to experimental characterization of living tissues. We will further develop appropriate mathematical
models that allow us to computationally predict the tissue response based on these proxy
materials. Collectively, we will provide a catalogue of biopolymeric proxy materials for different
soft tissues with corresponding modeling approaches. As a prospect, this will significantly facilitate
the choice of appropriate materials for 3D biofabrication of artificial organs, as well as modeling
approaches for predictive simulations. These form the cornerstone of advanced medical
treatment strategies and engineering design processes, leveraging virtual prototyping.
Multiscale modeling of nervous tissue: comprehensively linking microstructure, pathology, and mechanics
(FAU Funds)
Modeling and computation of growth in soft biological matter
(Third Party Funds Single)
Funding source: DFG-Einzelförderung / Sachbeihilfe (EIN-SBH)